Scientists look inside antimatter:
Going where no one has gone before
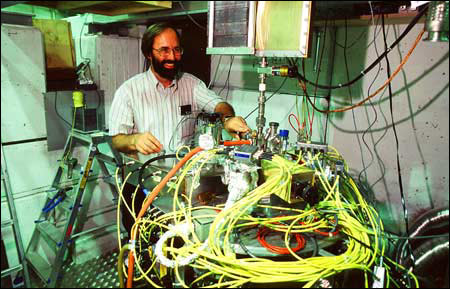
The Starship Enterprise is propelled through the universe of science fiction by a rocket fuel that combines ordinary matter and antimatter. When the two meet, they annihilate each other in a burst of energy that thrusts the starship from galaxy to galaxy.
Back in the 21st century, however, physicists still struggle with the problems of making antimatter and finding a container to put it in. This month, a Harvard-led research team published two reports in the journal Physical Review Letters that describe a giant step in this direction. For the first time, antimatter atoms have been slowed down enough to look inside them.
“We have obtained the first glimpse inside an antihydrogen atom, and this is a significant step on the way to precision measurements that will allow matter/antimatter comparisons to be made,” says Gerald Gabrielse, professor of physics at Harvard and leader of the research team. Such comparisons could show why antimatter and matter have not destroyed each other; in other words why there’s a universe at all. They also might cause physicists to scrap all their theories of how the universe operates.
Although no one is making any claims at this point, practical applications might come from the research. Culture-boggling devices like the laser and transistor emerged from efforts to solve basic physics problems, and the quest for an antimatter container has already produced new methods of analyzing medical drugs and ways to better shield medical imaging equipment from stray magnetic fields.
Looking for differences
According to the most accepted theories, a stupendous explosion (the big bang theory), in which the universe began some 15 billion years ago, should have yielded an equal amount of antimatter and matter. The two are mirror images of each other; that is, for every subatomic particle of matter there exists one of antimatter, but the latter has a reversed electric charge and spins in opposite directions. Antiprotons have a negative charge; antielectrons have a positive charge. These two get together to form antimatter atoms.
So where have all the antiatoms gone? Matter and antimatter should have annihilated each other and the universe disappeared in a great puff of energy before it got started. Obviously, that didn’t happen, and why is one of the biggest mysteries of science.
“If the structure of an antimatter atom differs from that of an ordinary atom by as little as one part in a hundred billion, that could suggest an important new route towards solving the mystery,” Gabrielse notes.
The easiest kind of antiatom to make is a hydrogen atom because it consists of only one antiproton and one antielectron, known as a positron. By the late 1980s, both types of antimatter had been made, so Gabrielse decided he would try to put them together. Such experiments cost a lot of money, so Gabrielse faced the task of raising funds. He sought approval for three grant applications from the head of the physics department at the University of Washington in Seattle where he was a postdoctoral student. His boss told him, “It will never fly,”
“Let me try,” Gabrielse insisted.
The agencies that he approached for funding asked him if he had approval to do the experiments at the Center for European Nuclear Research (CERN) near Geneva, Switzerland, the only place equipped to make enough antiprotons and positrons. When he asked for approval from CERN, they wanted to know if he had the money. He had to admit he did not.
It took a while, but with the help of colleagues from Germany, Gabrielse developed techniques to capture speeding antiprotons and slow them enough to make antiatoms. In 1996, two other research groups had actually created a few atoms of antihydrogen, but these zipped through their instruments at close to the speed of light, too fast to be measured.
When he and his colleagues slowed antiprotons enough, Gabrielse expanded his team to include other physicists from Germany, Switzerland, and Canada. Together they formed a new group called ATRAP (antihydrogen trap). Another collaboration of 39 physicists from 10 institutions, mostly in Europe, put together a competing group called ATHENA.
Cooling slows speeding particles, so ATRAP researchers dropped temperatures in their trap to about 469 degrees below zero Fahrenheit. Then they decided to run the cold antiprotons through a cloud of positrons. “Slowing antiprotons by colliding them with much lighter positrons is like slowing a car by driving it through a room full of pingpong balls,” Gabrielse explains. If you do it right the antiparticles slow down enough for some of them to stick together and form antiatoms.
What does it matter
Gabrielse describes the competition between ATRAP and ATHENA as “friendly,” but each team wanted to be the first to produce cold antihydrogen. ATRAP’s “star player” was a so-called “nested Penning trap,” invented to make the slowed antiprotons and positrons interact in a way that would unite them into antihydrogen. In August, the ATHENA team told Gabrielse that it had duplicated his device.
Gabrielse assumed that ATHENA would next attempt do what ATRAP was trying to do, improve the detection process before making an announcement that antihydrogen had, at last, been made and cooled to the point where it could be compared with hydrogen. But in September, ATHENA publicly announced that it had become the first group to tame antimatter. “I was frankly devastated” by the announcement, Gabrielse admits.
However, the game was not over. The ATHENA group had looked for evidence that pairs of positrons and antiprotons coming from the trap had destroyed themselves in close proximity and within five-millionths of a second of each other. When that happened, they counted it as an antihydrogen annihilation. But there is no good way to determine which particles came from antihydrogen and which had just come out of the trap alone.
ATRAP had lost the race to publication, but it had “antiaces” up its sleeve. Its detection method offered surer evidence of cold antihydrogen, as well as a first look into the internal structure of antiatoms. The group had installed a strong electric field at the mouth of the trap so that only antihydrogen could enter. Then the electric field tore the positrons out of the antiatoms, ripping the hard-earned antihydrogen in two. The freed antiprotons then were trapped and counted. Thus, Gabrielse comments “we know that every pair of antiprotons and positrons we get comes from an antihydrogen atom. There are no false signals, no background noise, no static.”
“It’s like putting antihydrogen atoms next to a battery,” Gabrielse continues. “The positive terminal attracts positrons, and the negative terminal draws antiprotons. How much voltage it takes to pull the atoms apart provides the first look ever into the internal structure of antihydrogen.”
The ATRAP look revealed what the ATHENA apparatus could not, that the positrons orbited further away from the antiprotons than expected. This means that the antiatoms are in an excited state, and they must be “calmed down” before the key comparisons can be made with everyday hydrogen.
“We’re working on this now,” Gabrielse says. “It’s our next challenge.”
Getting real
Suppose no differences between antihydrogen and hydrogen are found? “That certainly would be less fun than finding one, and it’s a real possibility,” Gabrielse admits. The mystery of what happed to antimatter would remain unsolved. Theories of how the universe works, as unsatisfactory as they are, would not be improved.
“We’re fishing in an interesting pond,” Gabrielse comments. “Any new thing that we hook is potentially of great importance.” That probably will not include new rocket fuels for spaceships, but such fishing has produced some interesting catches. The trap ATRAP designed to hold antimatter in a magnetic grip is being used to hold drug compounds so they can be analyzed more precisely than heretofore possible. This could lead to more potent drugs with fewer side effects.
A decade ago, Gabrielse and his colleagues developed a new device to shield traps from stray magnetic fields (see sidebar). Today, such shields can be purchased commercially to protect equipment that makes magnetic resonance images of the brain and body. Hospitals can use them to shield their imaging equipment from magnetic disturbances caused by elevators going up and down in a building.
“If you push reality hard enough,” Gabrielse says, “good things always come out of it.”